Resource Library
Your gateway to resources for and about gifted students.
Tips for Students: Order and Chaos: The Physics of Energy & Entropy
The following article expands on highlights and insights from one…
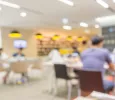
Tips for Parents: Fostering Regulation and Resilience in PG and Neurodivergent Children
The following article expands on highlights and insights from one…
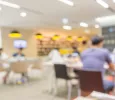
Tips for Parents: Bridging to Homeschooling: A Personalized Pathway to Learning Success
The following article expands on highlights and insights from one…
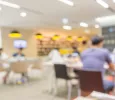
8 Myths About Gifted Students Debunked
The concept of “giftedness” tends to be misunderstood. This lack…
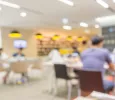
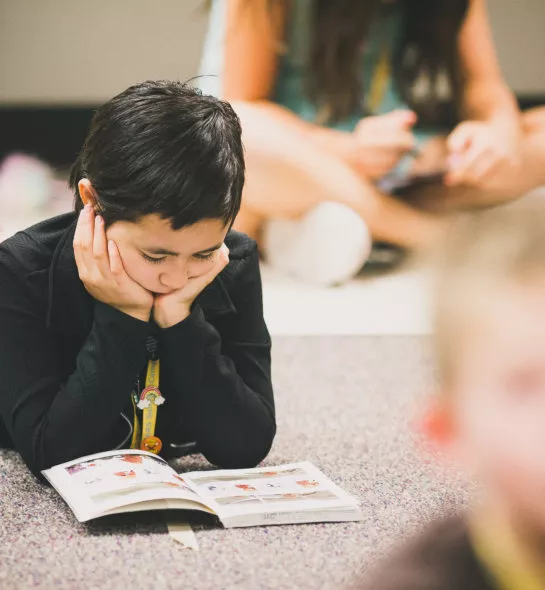
Free Resources and Guides
Families can find gifted resources by browsing popular topics and issues in the gifted community. We also have extensive guides written by the Davidson Institute professionals have been designed to assist families with gifted students.
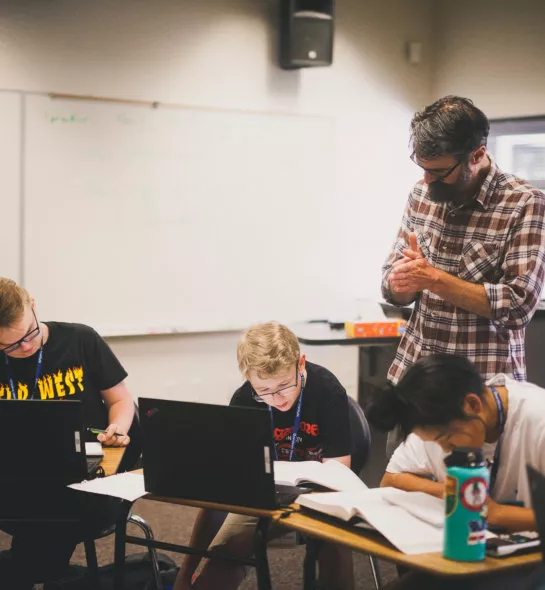
Support for Educators
Join the online community of professionals committed to meeting the unique needs of highly gifted students.
Davidson Institute Spotify Playlists
Now you can take Davidson with you! We’ve curated topic-specific playlists of the podcast episodes our Family Services Team has been listening to and recommending to families. Now, they are all in one convenient spot, so just follow us on Spotify, and listen along on your schedule!